Modern materials science has allowed us to enhance and modify the physical properties of materials to satisfy our ever-expanding technological needs. Modern materials, however, lack the complex functionalities common in biological systems, such as the self-healing that our skin and bones perform so efficiently.
The Pikul Research Group seeks to develop multifunctional materials systems to achieve mechanical and chemical properties not available in natural or man-made engineering materials. Our toolkit includes expertise in electrochemistry, bottom-up fabrication methods, and the characterization of materials from the mesoscale to the nanoscale.
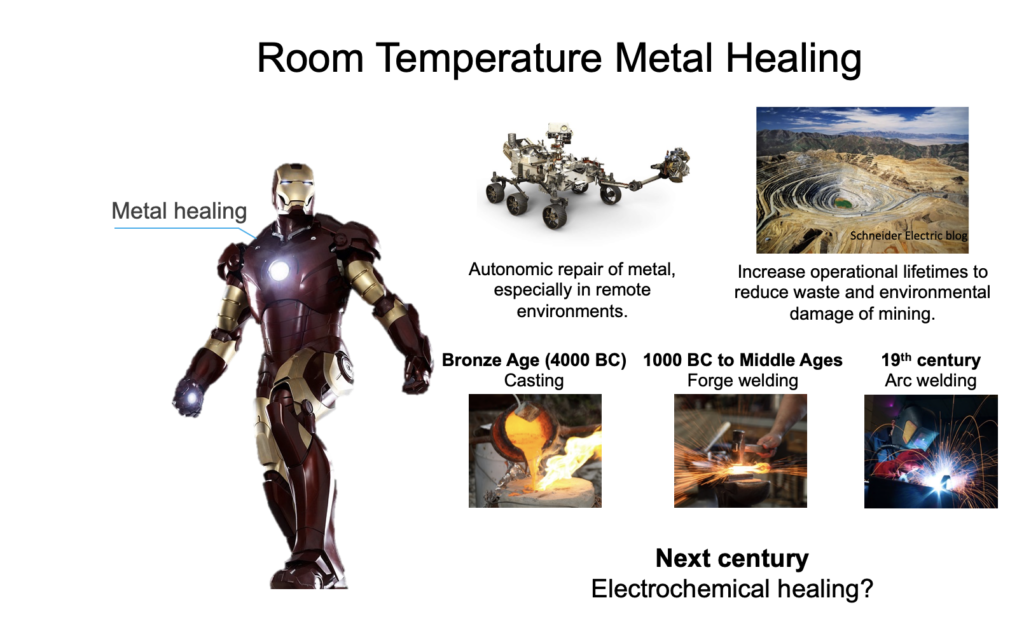
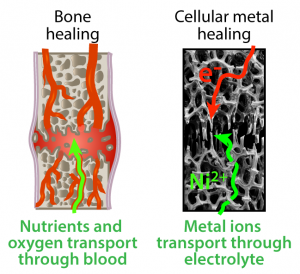
Structural materials in living organisms can heal fractures at or near room temperature, whereas healing fractured metal requires high temperatures and large energy inputs. By taking inspiration from the transport-mediated healing of bone, we demonstrated rapid, effective, and low-energy healing of metal at room temperature using the electrochemical transport of metal ions within a metallic cellular material.
To realize electrochemical healing, cellular nickel was conformally coated with an insulating and chemically-inert polymer, which allowed healing only at fracture sites where the polymer coating cracked and exposed the underlying nickel. After immersing the cellular nickel into an external electrolyte nickel ions could be transported from an anode to the fractured nickel struts. The combination of ion migration, fast ion diffusion, and the cellular structure enabled 100% strength recovery of 1.6 mm thick fractured samples after as little as 1500 J and 4 h of potentiostatic healing at room temperature. Healed samples fully recovered their strength after being loaded to within 1% strain of total failure, which corresponded to a 350% increase in the fractured nickel strength. In addition, applying an electric current to the samples before catastrophic failure would strengthen the areas which has the largest stress concentrations and increase the samples resistance to subsequent failure.
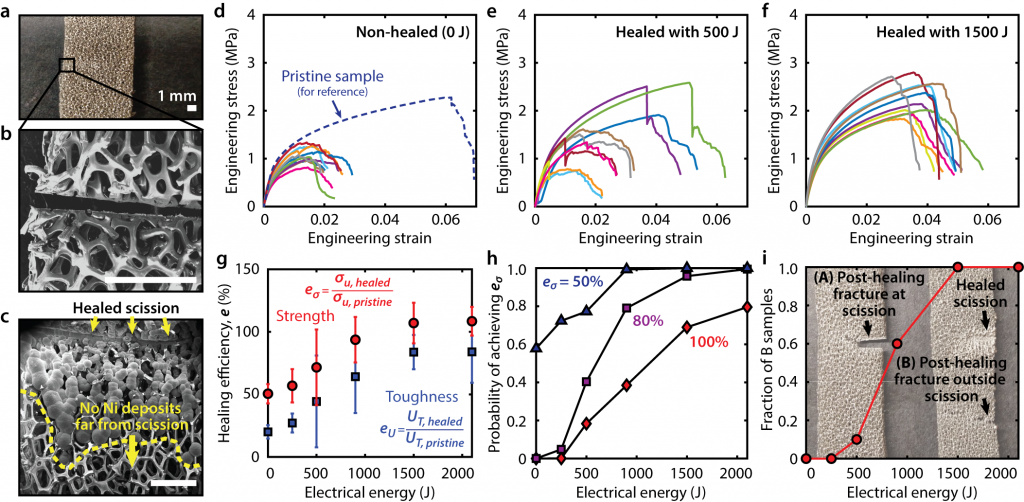
In addition, electrochemical healing of cellular nickel at room temperature requires lower energy input than most metal healing techniques. The samples presented here could be healed 50-100 times with an average cell phone battery.
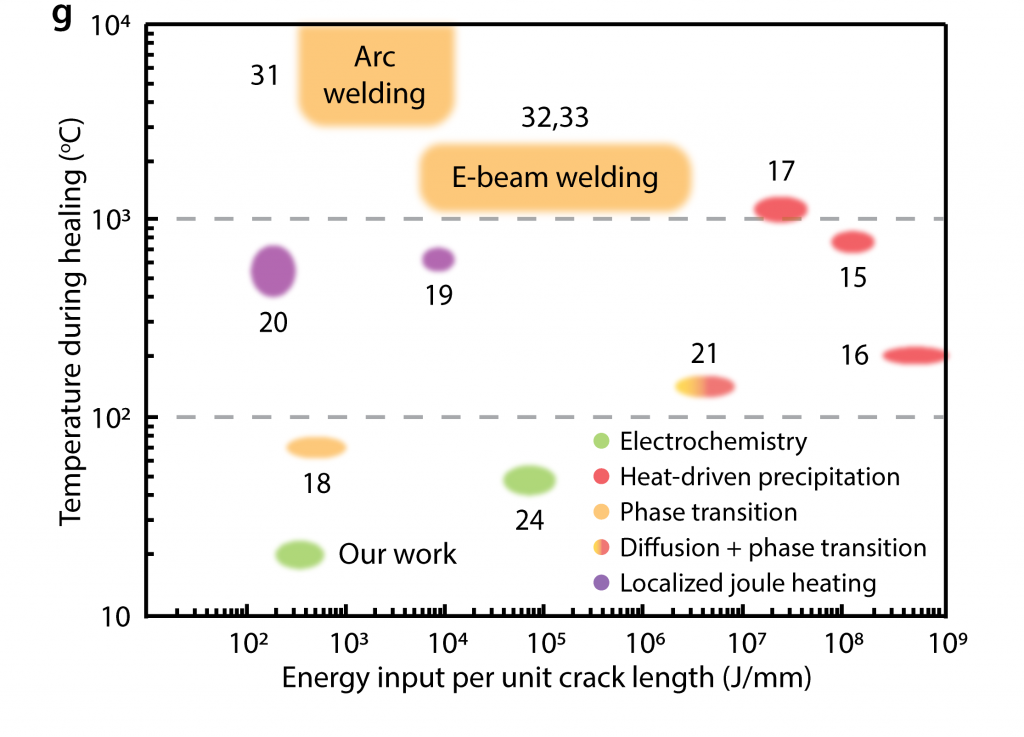
Publications:
[29] Zakaria Hsain, Zhimin Jiang, and James H. Pikul, “Enabling effective electrochemical healing of structural steel”, Multifunctional Materials, vol. 4, no. 2, 024004, 2021.
[21] James H. Pikul, Jeffrey W. Long, “Architected Materials for Advanced Electrochemical Systems“, MRS Bulletin, vol. 44, no. 10, October 2019.
[20] Zakaria Hsain, James H. Pikul, “Low-energy Room-temperature Healing of Cellular Metals“, Advanced Functional Materials, pp. 1905631, August 2019.