Modern robots lack the multifunctional, interconnected systems found in living organisms and, consequently, exhibit reduced efficiency and autonomy. Energy storage systems are among the most visible limitations to robot autonomy, but the basic design of battery cells has undergone relatively few changes since the late 1800’s, despite the dramatic advances in chemistry and material processing. In addition, emerging energy storage applications are placing increased demands on the mechanical, thermal, and chemical properties of batteries, as well as requiring improved energy storage capabilities. We seek to create new classes of energy storage devices with a focus towards robotics applications by realizing new designs that take advantage of modern robotic capabilities and increased autonomy.
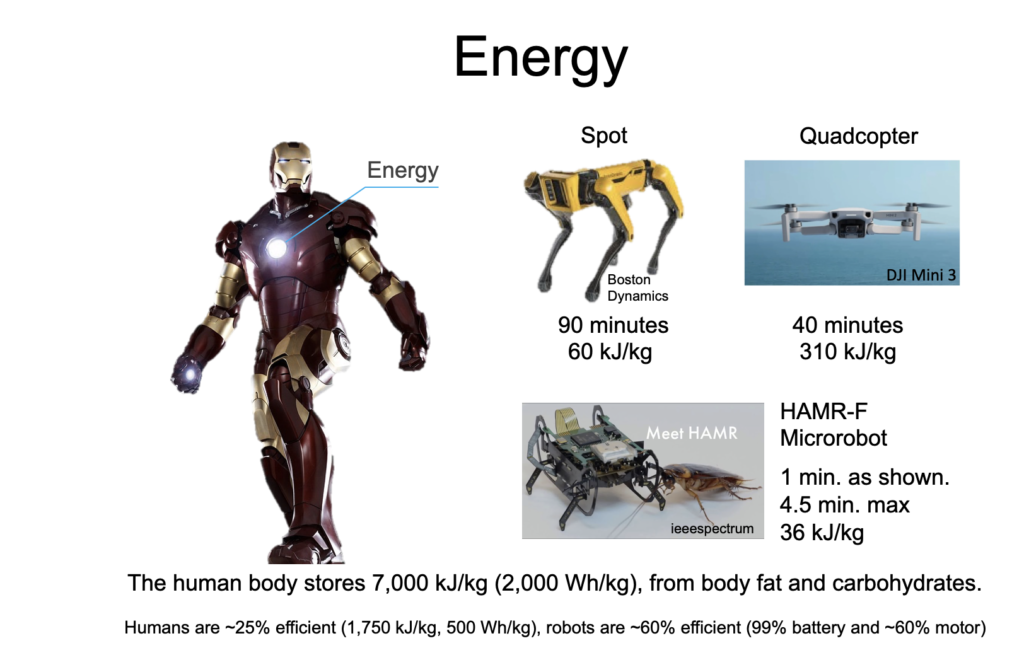
- Metal consuming robots
In this work, we show that semi-solid hydrogel electrolytes with oxygen reduction cathodes, a device we call a metal-air scavenger (MAS), can electrochemically extract energy from external metals to achieve high energy and power density, combining the benefits of batteries and energy harvesters, see ref. [23]. The MAS facilitates electrochemical reactions that occur in metal-air batteries, but the anode and cathode are both external to the MAS. We show that, when stationary, a MAS can extract 159 mAh/cm2, 87 mAh/cm2, and 179 mAh/cm2 from aluminum, zinc, and stainless-steel surfaces at up to 130, 81, and 25 mW/cm2 power densities. A principal advantage of the MAS is that it can continue to power a device as it travels across a metal surface, so that the total mass and volume of metal oxidized are many multiples of the mass and volume of the device. This property of the MAS breaks energy storage scaling laws by allowing small robots and electronics to extract energy from large volumes of energy dense material without having to carry the material on-board. Finally, we demonstrate the utility of a traveling MAS by powering a 5 x 3 x 1 cm electric vehicle on an aluminum surface with a 2 x 3 x 0.2 cm MAS. When storing excess electrolyte, a traveling MAS can achieve 3,082 Wh/kgMAS energy densities on aluminum surfaces, which is more than 2 times larger than the best aluminum-air batteries fabricated to date, and 12 times greater than commercial lithium-ion batteries (243 Wh/kg).
Computer-free autonomous decision making based on environmental cues would provide exciting alternatives to classic control systems for robots and smart materials. Although this functionality has been studied in microswimmers and active colloids where energy in the surrounding liquid is prevalent, there are no devices that can provide sufficient power from environmental chemicals to move and steer larger scale robots and vehicles in dry environments. We have recent work in ref. [27] that overcomes this limitation with an Environmentally Controlled Voltage Source (ECVS, similar to the MAS above) that, when directly attached to electric motors on a vehicle, can increase the energy available to the vehicle and provide computer-free autonomous navigation towards chemical fuels in the environment and away from hazards. The ECVS uses electrochemistry to extract power from the chemical fuels and the vehicle avoids hazards that reduce the output voltage or electrochemical kinetics. Two ECVSs can also be arranged in series or parallel to perform logical functions based on the chemicals in contact with the ECVSs. This work presents a new method to simultaneously steer and power vehicles and robots without computers by directly responding to a wide variety of chemical fields in their environment using electrochemistry.
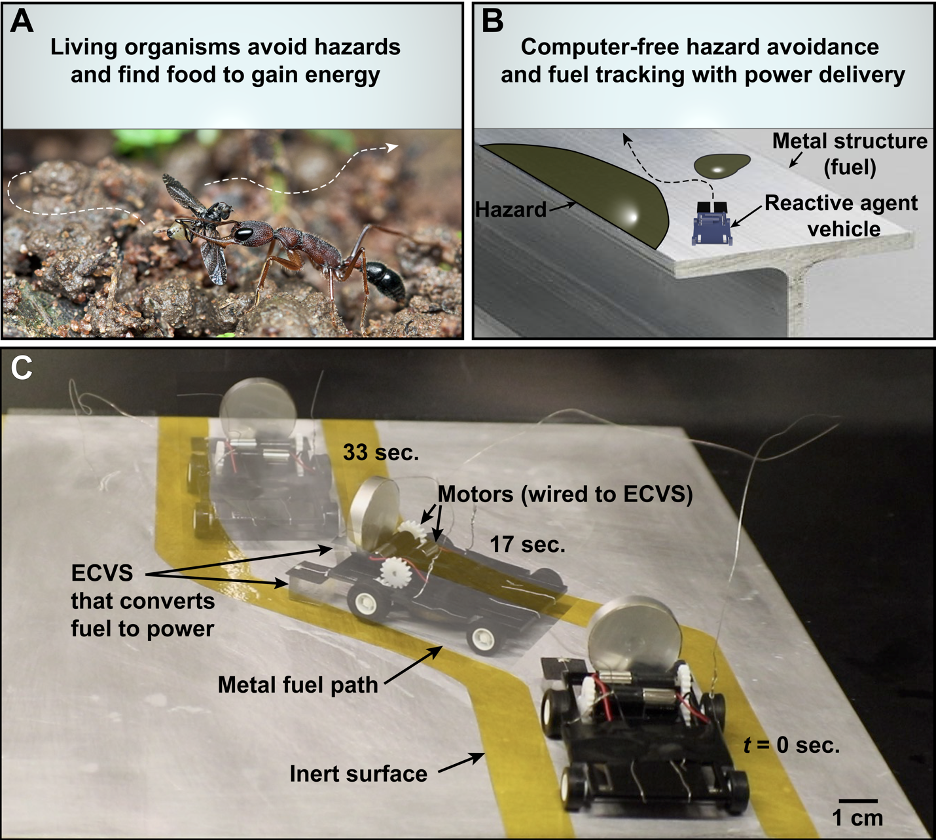
Figure 1. Autonomous navigation. (A) An illustration of an ant that avoids hazards and follows food to gain energy. The photo is modified from “Jerdon’s jumping ant with prey” by vipin baliga, licensed under CC BY-NC-SA 2.0. (B) A schematic of a synthetic analog consisting of a vehicle that navigates along a metal fuel source while avoiding hazards. (C) Sequential images of a reactive agent vehicle following a metal fuel path without computers. From ref. [27]
2. Synthetic multifunctional circulatory systems
In this project, we presented a synthetic, energy-dense circulatory system embedded in an untethered, aquatic soft robot. Modeled after redox flow batteries, this vascular system combines the functions of hydraulic force transmission, actuation, and energy storage into a single integrated design that geometrically increases the energy density of the robot to enable long duration operation. The fabrication techniques and compliant materials used in its construction allow the vascular system to be shaped into complex form factors that continuously deform with robot movement. The complete robotic fish has a system energy density of 53 J g-1, a 4X gain over the same fish with only lithium ion batteries, and can swim for long durations (max theoretical operating time = 36.7 hours) at 1.56 body lengths per minute, up stream. This use of electrochemical energy storage in hydraulic fluids could facilitate increased energy density, autonomy, efficiency, and multifunctionality in future robot designs. Read more about it in ref. [19]
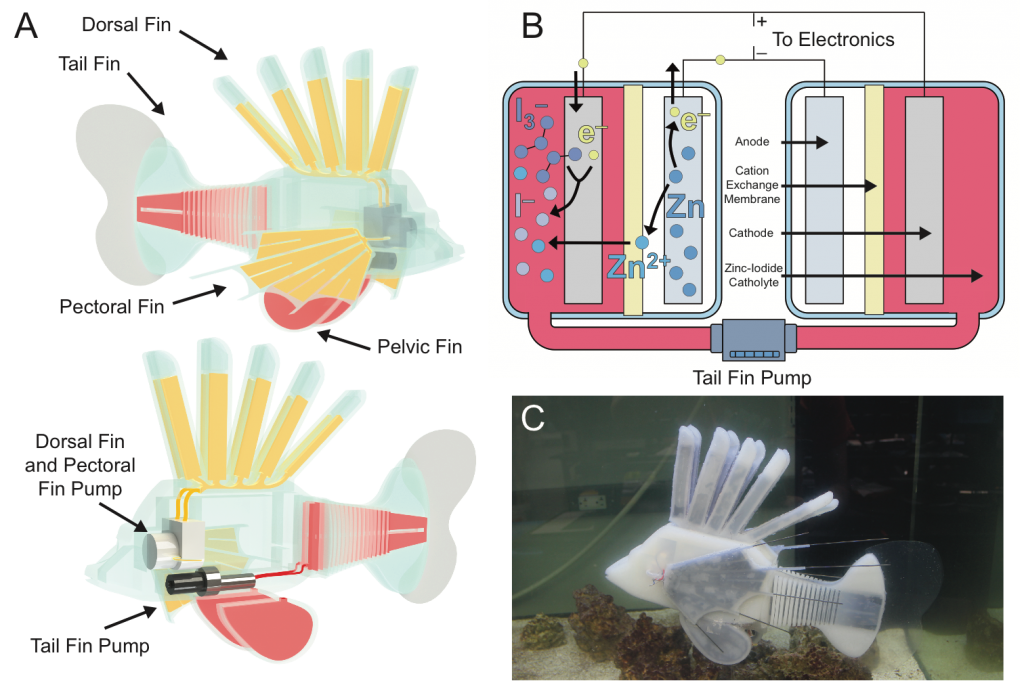
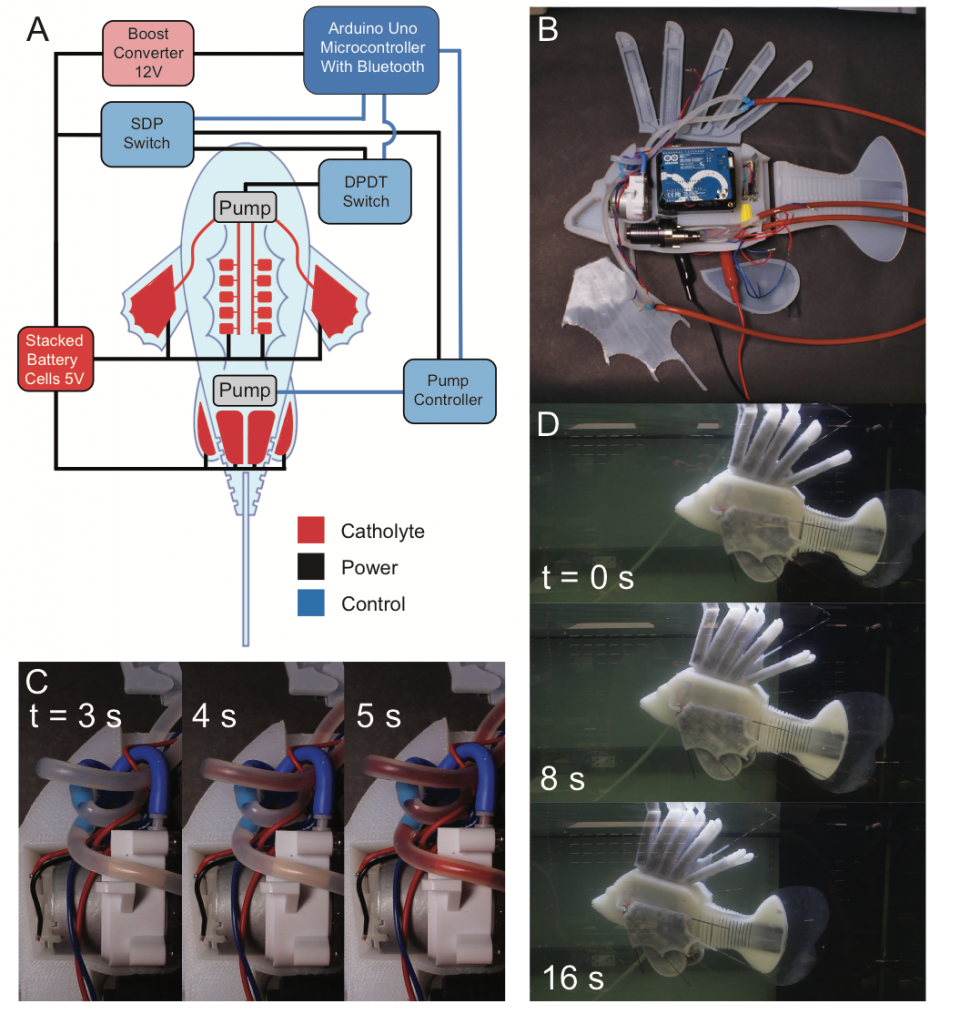
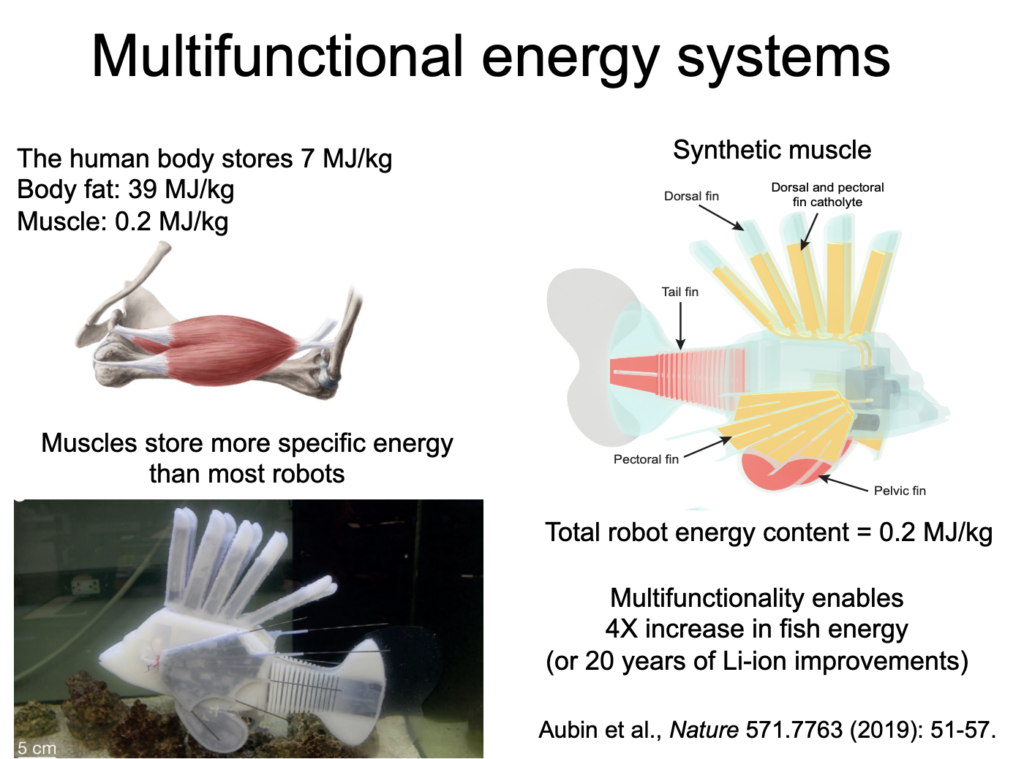
3. Microbatteries for microrobots
Billions of internet connected devices used for medicine, wearables, and robotics require microbattery power sources, but the conflicting scaling laws between electronics and energy storage have led to inadequate power sources that severely limit the performance of these physically small devices. We have developed a new design paradigm for primary microbatteries that drastically improves energy and power density by eliminating the vast majority of the packaging and through the use of high energy density anode and cathode materials. Figure 5 and 6 shows the design and performance of these microbatteries from ref. [32]. These light (50-80 mg) and small (20-40 μL) microbatteries are enabled though the electroplating of 130 µm thick 94% dense additive-free and crystallographically oriented LiCoO2 onto thin metal foils, which also act as the encapsulation layer. These devices have 430 Wh kg-1 and 1050 Wh L-1 energy densities, 4 times the energy density of previous similarly sized microbatteries, opening up the potential to power otherwise unpowerable microdevices.
Increasing the density and thickness of battery electrodes can lower costs, ease manufacturing, and increase energy density; however, existing electrode architectures cannot simultaneously enable thick and dense electrodes with good power densities. In particle-based architectures, long range lithium-ion transport primarily occurs through the electrolyte, but electrolyte transport pathways disappear as electrode density approaches 100%. The loss of these transport pathways leads to dramatic capacity reductions at moderate discharge rates and has set minimum porosity limits for commercial cells. We have also examined transport through three thick and dense cathode architectures to understand the interdependent impact of inter-particle interfaces, continuous diffusion lengths, solid volume fraction, solid diffusivity, cathode thickness, and discharge rate on areal capacity. This work is published in ref. [35]. We demonstrate the advantages of continuous cathode architectures and show how the combination of high diffusivity and continuous solid diffusion pathways can yield 65% increases in areal capacity over a conventional, particle-based electrode at 85% solids volume fraction. Figure 7 summarized the results. We also show that combining these high-diffusivity, continuous cathode architectures with solid electrolytes can overcome some of the inherent limitations of current solid-state battery designs.
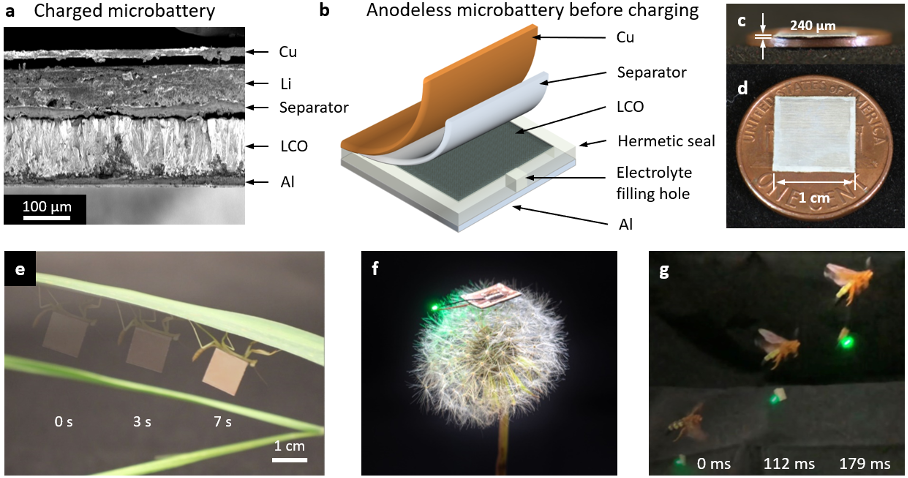
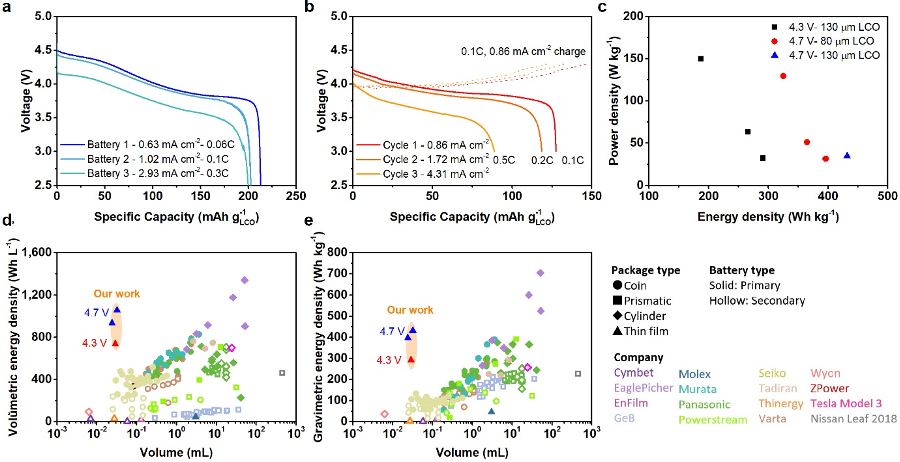
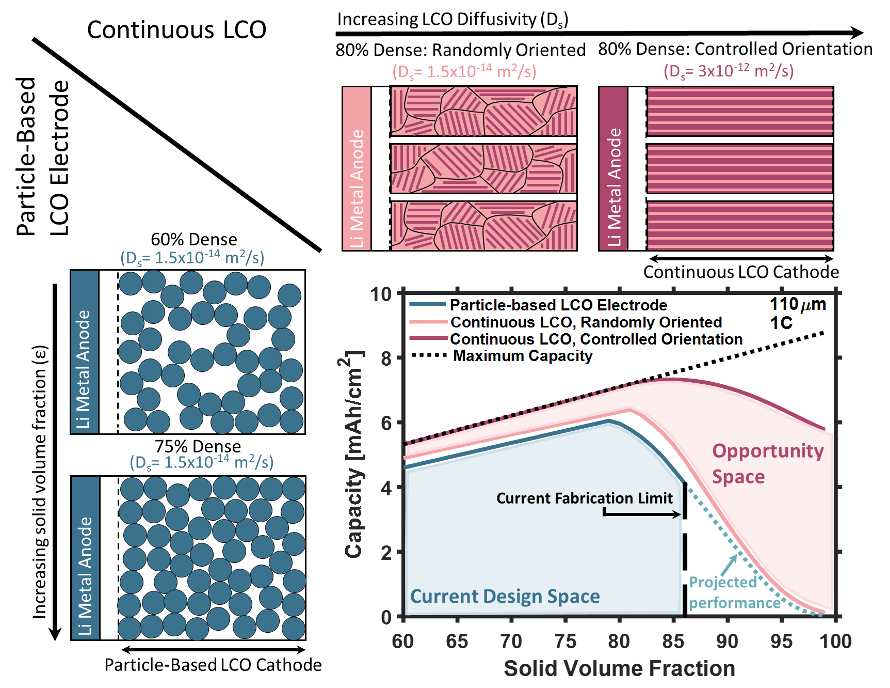
References:
Metal consuming robots:
[28] Min Wang, Andrew C. Meng, Jintao Fu, Alexandre C. Foucher, Rui Filipe Serra Maia, Eric A. Stach, Eric Detsi, and James H. Pikul, “Surface Facet Engineering in Nanoporous Gold for Low-Loading Catalysts in Aluminum-Air Batteries”, ACS Applied Materials and Interfaces, 2021.
[27] Min Wang, Yue Gao, and James H. Pikul, “Computer-free autonomous Navigation and Power Generation Using Electro‐Chemotaxis”, Advanced Intelligent Systems, 2000255, 2021
[23] Min Wang, Unnati Joshi, James H. Pikul, “Powering electronics by scavenging energy from external metals” ACS Energy Letters, vol. 5, no.3, pp. 758-765, 2020.
Synthetic multifunctional circulatory systems
[19] Cameron A. Aubin, Snehashis Choudhury, Rhiannon Jerch, Lynden A. Archer, James H. Pikul, Robert F. Shepherd, “Electrolytic vascular systems for energy-dense robots“, Nature, vol. 571, June 2019.
Microbatteries
[35] Alissa C Johnson, Adam J Dunlop, Ryan R Kohlmeyer, Chadd T Kiggins, Aaron J Blake, Soninka V Singh, Evan M Beale, Beniamin Zahiri, Arghya Patra, Xiujun Yue, John B Cook, Paul V Braun, James H Pikul, “Strategies for approaching one hundred percent dense lithium-ion battery cathodes” Journal of Power Sources, 532, 231359, 2022.
[32] Xiujun Yue, Alissa C. Johnson, Sungbong Kim, Ryan R. Kohlmeyer, Arghya Patra, Jessica Grzyb, Akaash Padmanabha, Min Wang, Zhimin Jiang, Pengcheng Sun, Chadd T. Kiggins, Mehmet N. Ates, Sonika V. Singh, Evan M. Beale, Mark Daroux, Aaron J. Blake, John B. Cook, Paul V. Braun, James H. Pikul, “A nearly packaging-free design paradigm for light, powerful, and energy dense primary microbatteries”, Advanced Materials, 2101760, 2021.
[24] Xiujun Yue, Jessica Grzyb, Akaash Padmanabha, and James H. Pikul. “A Minimal Volume Hermetic Packaging Design for High-Energy-Density Micro-Energy Systems” Energies vol. 13, no. 10, pp. 2492, 2020.
[22] Michael Synodis, James Pikul, Sue Ann Bidstrup Allen, and Mark G. Allen. “Vertically integrated high voltage Zn-Air batteries enabled by stacked multilayer electrodeposition“, Journal of Power Sources, vol. 449 pp. 227566, 2020.
[16] James H. Pikul, Hailong Ning, “Powering the internet of things”, Joule, vol. 2, pp. 1036–1038, June 20, 2018.
[11] James H. Pikul, Paul V. Braun, and William P. King. “Performance Modeling and Design of Ultra-High Power Microbatteries” Journal of The Electrochemical Society, vol. 164.11, pp. E3122-E3131,2017.
[10] James H. Pikul, Jinyun Liu, Paul V. Braun, William P. King, “Integration of high capacity materials into interdigitated mesostructured electrodes for high energy and high power density primary microbatteries” Journal of Power Sources, vol. 315, pp. 308-315, 2016.
[9] Hailong Ning, James H. Pikul, Runyu Zhang, Xuejiao Li, Sheng Xu, Junjie Wang, John A. Rogers, William Paul King, and Paul V. Braun, “Holographic Patterning of High Performance on-chip 3D Lithium-ion Microbatteries”, Proceeding of the National Academy of Sciences, vol. 112, no. 21, pp. 6573-6578, 2015.
[6] James H. Pikul, Huigang Zhang, Jiung Cho, Paul V. Braun, and William P. King, “High power lithium ion micro batteries from interdigitated three-dimensional bicontinuous nanoporous electrodes”, Nature Communications, vol. 4, pp. 1732, 2013